A long while ago I promised to take you from the action by the modular group
Speaking of dessins d’enfant, let me point you to the latest intriguing paper by Yuri I. Manin and Matilde Marcolli, ArXived a few days ago Quantum Statistical Mechanics of the Absolute Galois Group, on how to build a quantum system for the absolute Galois group from dessins d’enfant (more on this, I promise, later).
Where were we?
We’ve seen natural one-to-one correspondences between (a) points on the projective line over
How to get from there to a dessin d’enfant?
The short answer is: it’s all in Ravi S. Kulkarni’s paper, “An arithmetic-geometric method in the study of the subgroups of the modular group”, Amer. J. Math 113 (1991) 1053-1135.
It is a complete mystery to me why Tatitscheff, He and McKay don’t mention Kulkarni’s paper in “Cusps, congruence groups and monstrous dessins”. Because all they do (and much more) is in Kulkarni.
I’ve blogged about Kulkarni’s paper years ago:
– In the Dedekind tessalation it was all about assigning special polygons to subgroups of finite index of
– In Modular quilts and cuboid tree diagram it did go on assigning (multiple) cuboid trees to a (conjugacy class) of such finite index subgroup.
– In Hyperbolic Mathieu polygons the story continued on a finite-to-one connection between special hyperbolic polygons and cuboid trees.
– In Farey codes it was shown how to encode such polygons by a Farey-sequence.
– In Generators of modular subgroups it was shown how to get generators of the finite index subgroups from this Farey sequence.
The modular group is a free product
with lifts of
As a result, any permutation representation of
Each white vertex has two (or one) edges connected to it and every black vertex has three (or one). These edges are the elements of
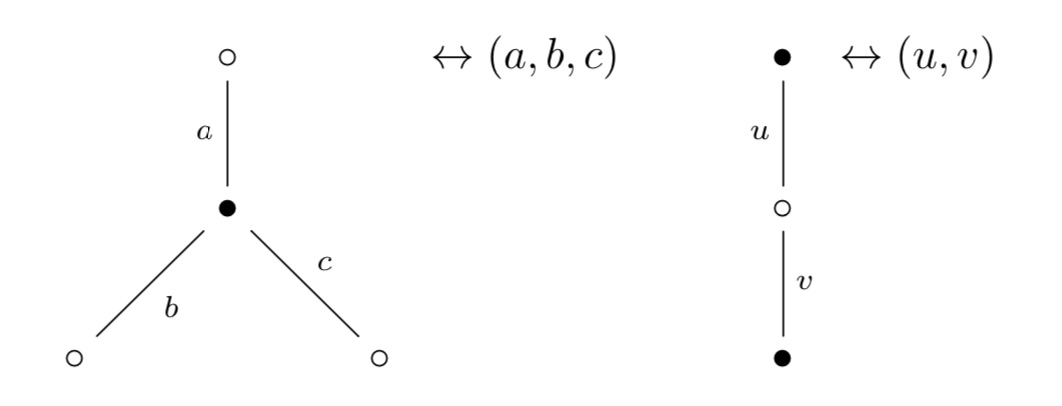
Clearly, if there’s just one edge connected to a vertex, it gives a fixed point (or 1-cycle) in the corresponding permutation.
The ‘monstrous dessin’ for the congruence subgroup
Kulkarni’s paper (or the blogposts above) tell you how to get at this picture starting from a fundamental domain of
Sage gives a nice image of this fundamental domain via the command
FareySymbol(Gamma0(n)).fundamental_domain()
Here’s the image for
.jpg)
The boundary points (on the halflines through
To get the dessin from this, let’s first look at the interior points. A white vertex is a point in the interior where two black and two white tiles meet, a black vertex corresponds to an interior points where three black and three white tiles meet.
Points on the boundary where tiles meet are coloured red, and after identification two of these reds give one white or black vertex. Here’s the intermediate picture
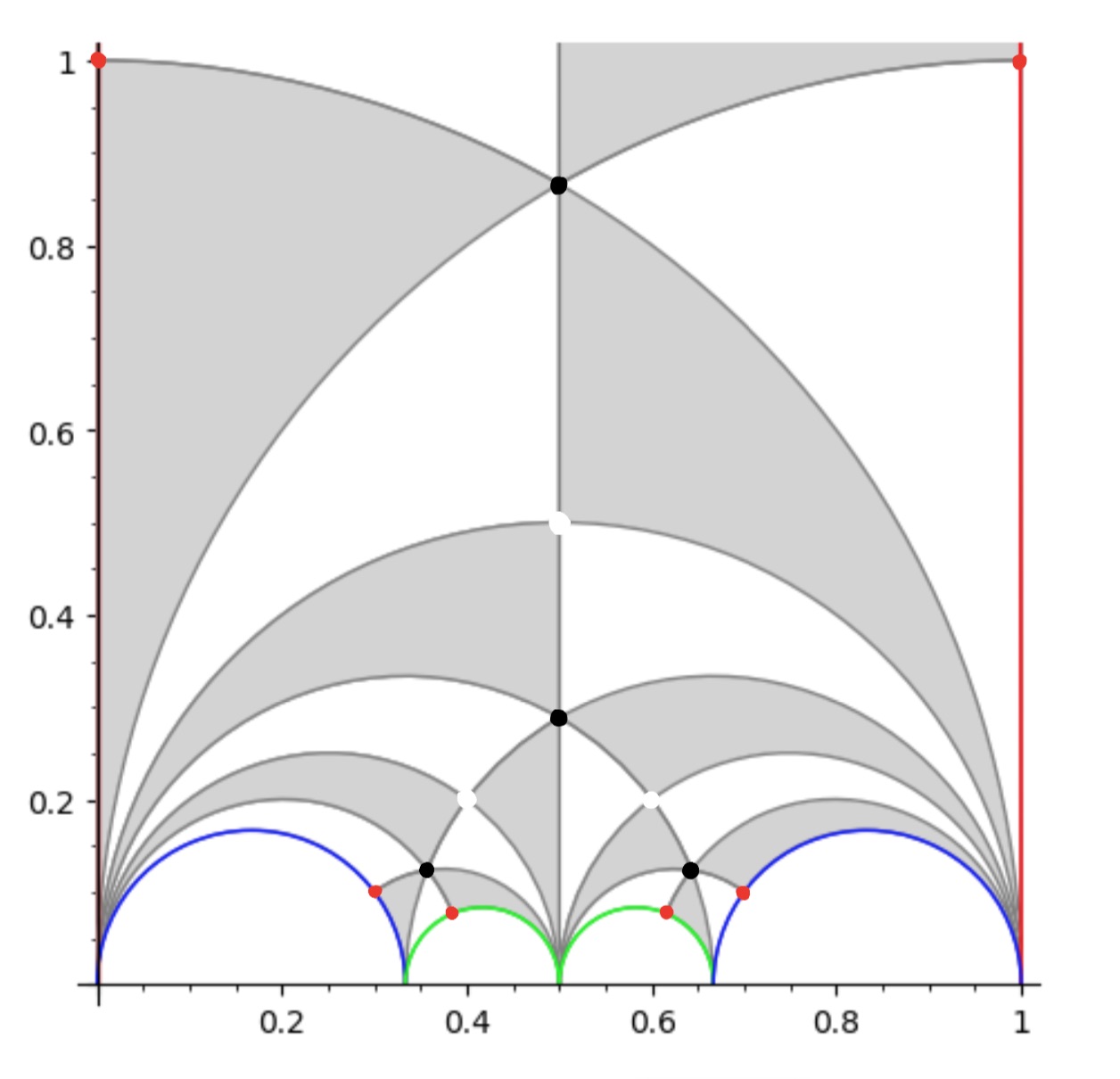
The two top red points are identified giving a white vertex as do the two reds on the blue half-circles and the two reds on the green half-circles, because after identification two black and two white tiles meet there.
This then gives us the ‘monstrous’ modular dessin for
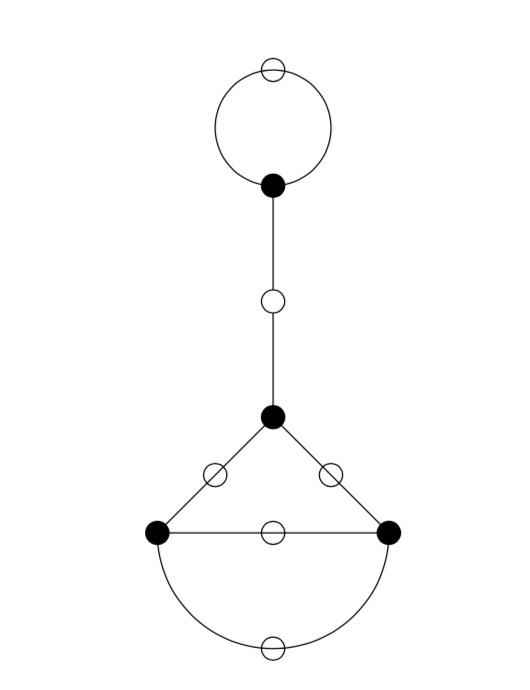
Let’s try a more difficult example:
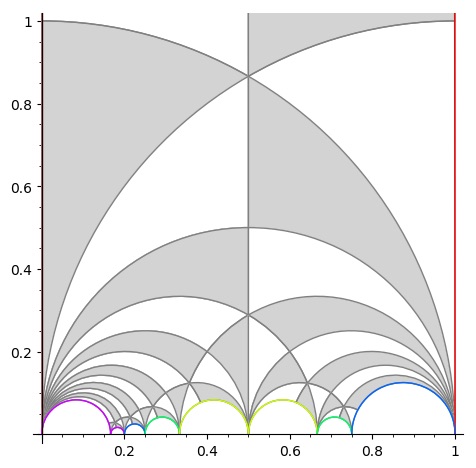
giving us the intermediate picture
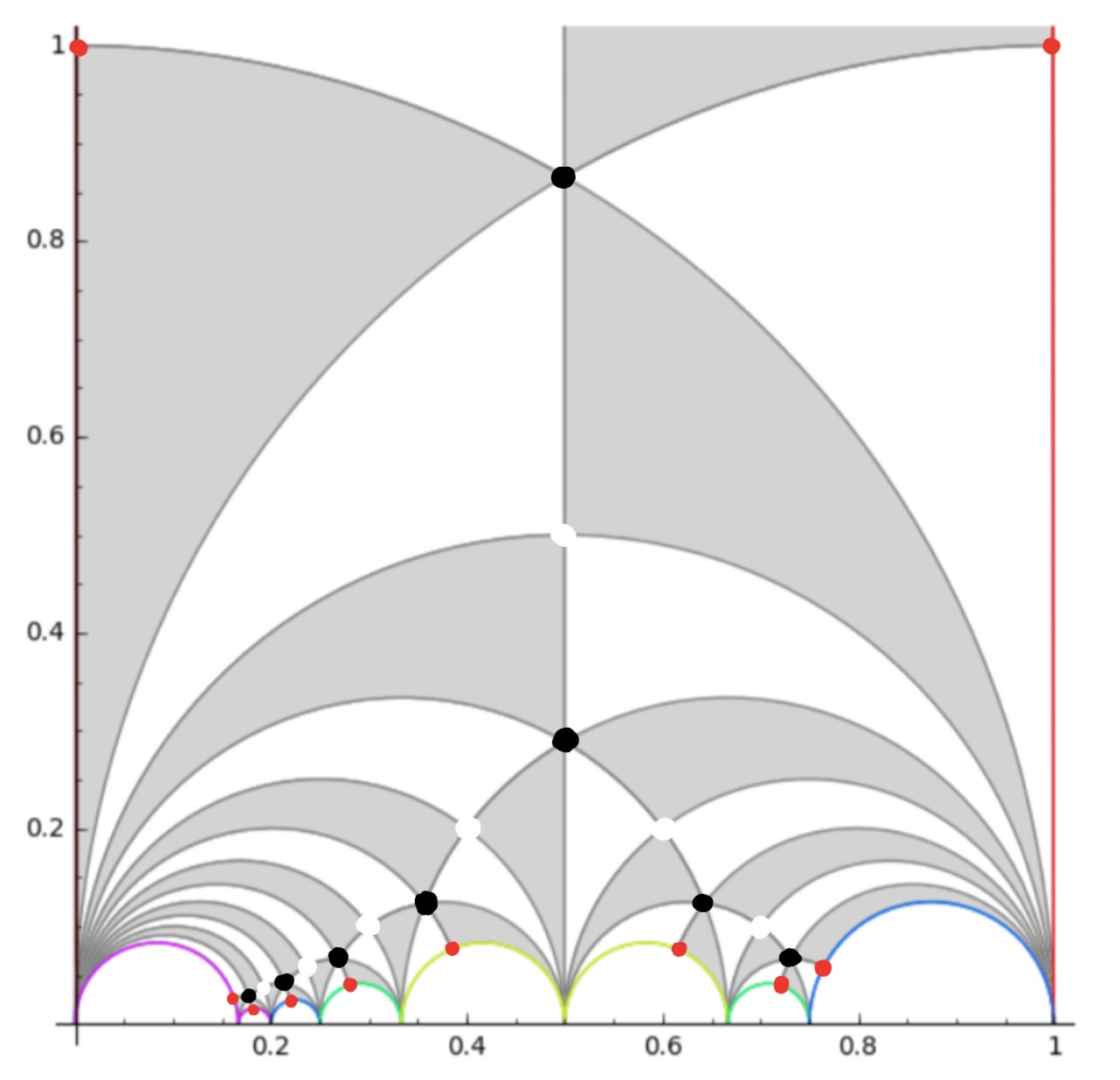
and spotting the correct identifications, this gives us the ‘monstrous’ dessin for
In general there are several of these 2-coloured graphs giving the same permutation representation, so the obtained ‘monstrous dessin’ depends on the choice of fundamental domain.
You’ll have noticed that the domain for
This is caused by Sage using the Farey-code
One of the nice results from Kulkarni’s paper is that for any
It would be nice to see whether using these symmetric Farey-codes gives other ‘monstrous dessins’ than in the THM-paper.
Remains to identify the edges in the dessin with the lattices at hyperdistance
Using the tricks from the previous post it is quite easy to check that for any
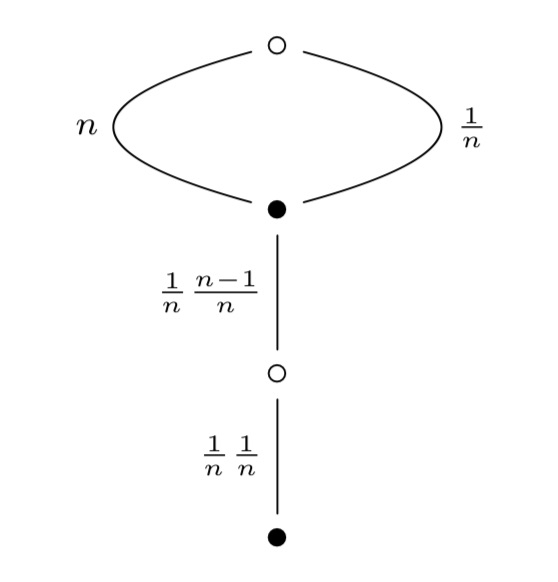
Let’s do a sample computation showing that the action of
and then, as last time, to determine the class of the lattice spanned by the rows of this matrix we have to compute
which is class