The Monster is the largest of the 26 sporadic simple groups and has order
808 017 424 794 512 875 886 459 904 961 710 757 005 754 368 000 000 000
= 2^46 3^20 5^9 7^6 11^2 13^3 17 19 23 29 31 41 47 59 71.
It is not so much the size of its order that makes it hard to do actual calculations in the monster, but rather the dimensions of its smallest non-trivial irreducible representations (196 883 for the smallest, 21 296 876 for the next one, and so on).
In characteristic two there is an irreducible representation of one dimension less (196 882) which appears to be of great use to obtain information. For example, Robert Wilson used it to prove that The Monster is a Hurwitz group. This means that the Monster is generated by two elements g and h satisfying the relations
Geometrically, this implies that the Monster is the automorphism group of a Riemann surface of genus g satisfying the Hurwitz bound 84(g-1)=#Monster. That is,
g=9619255057077534236743570297163223297687552000000001=42151199 * 293998543 * 776222682603828537142813968452830193
Or, in analogy with the Klein quartic which can be constructed from 24 heptagons in the tiling of the hyperbolic plane, there is a finite region of the hyperbolic plane, tiled with heptagons, from which we can construct this monster curve by gluing the boundary is a specific way so that we get a Riemann surface with exactly 9619255057077534236743570297163223297687552000000001 holes. This finite part of the hyperbolic tiling (consisting of #Monster/7 heptagons) we’ll call the empire of the monster and we’d love to describe it in more detail.
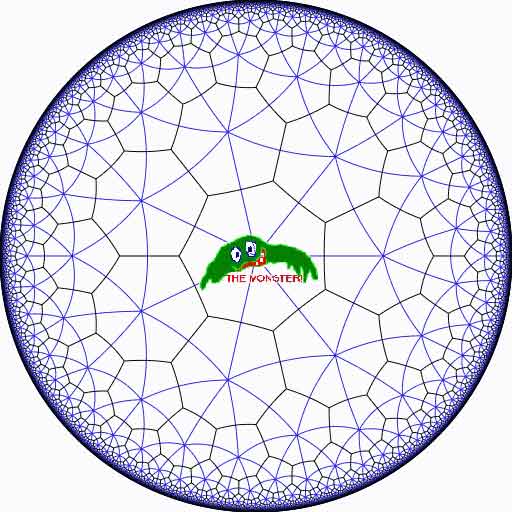
Look at the half-edges of all the heptagons in the empire (the picture above learns that every edge in cut in two by a blue geodesic). They are exactly #Monster such half-edges and they form a dessin d’enfant for the monster-curve.
If we label these half-edges by the elements of the Monster, then multiplication by g in the monster interchanges the two half-edges making up a heptagonal edge in the empire and multiplication by h in the monster takes a half-edge to the one encountered first by going counter-clockwise in the vertex of the heptagonal tiling. Because g and h generated the Monster, the dessin of the empire is just a concrete realization of the monster.
Because g is of order two and h is of order three, the two permutations they determine on the dessin, gives a group epimorphism
In noncommutative geometry, the group-algebra of the modular group
To make sense of this question, let us first consider the commutative equivalent : what does a point P see of a commutative variety X?
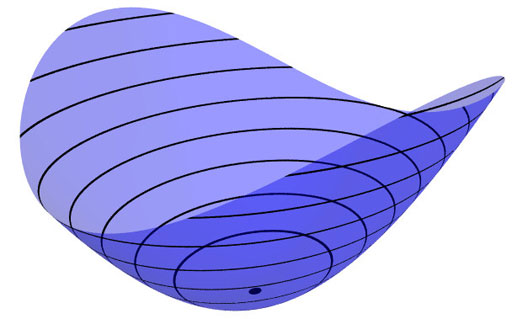
Evaluation of polynomial functions in P gives us an algebra epimorphism
Equivalently, we can view the point
This sounds pretty useless, but let us now consider higher-order formal neighborhoods. Call the affine scheme
The second neighborhood
These successive quotients by powers of the maximal ideal
and its inverse limit
In case P is a smooth point of X, then X is a manifold in a neighborhood of P and then this completion
Right, after this lengthy recollection, back to our question what does the monster see of the modular group? Well, we have an algebra epimorphism
and in analogy with the commutative case, all information the Monster can gain from the modular group is contained in the
where
As it is a hopeless task to determine the Monster-empire explicitly, it seems even more hopeless to determine the kernel
Here the details : the quotient
with exactly 194 components (the number of irreducible Monster-representations). For any
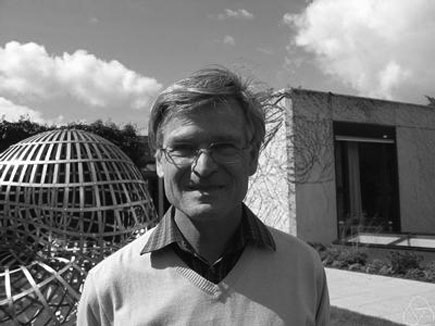
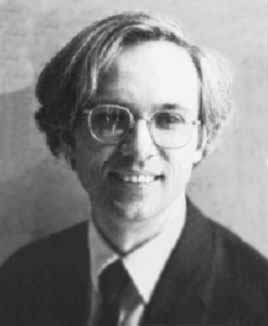
and applying the formal neighborhood theorem for formally smooth algebras (such as
where the right-hand side is the completion of the tensor-algebra (at the unique graded maximal ideal) of the
Okay, so what’s a bimodule over a semisimple algebra of the form
That is, any S-bimodule can be represented by a quiver (that is a directed graph) on k vertices (the number of matrix components) with a loop in vertex i corresponding to each simple factor of type (1) and a directed arrow from i to j corresponding to every simple factor of type (2).
That is, for the Monster, the bimodule
Using Morita equivalences and standard representation theory of quivers it isn’t exactly rocket science to determine that the number of arrows between the vertices corresponding to the irreducible Monster-representations
Now, I’ve been wasting a lot of time already here explaining what representations of the modular group have to do with quivers (see for example here or some other posts in the same series) and for quiver-representations we all know how to compute Ext-dimensions in terms of the Euler-form applied to the dimension vectors.
Right, so for every Monster-irreducible
Now the dimensions
So, for each of the 194 irreducible Monster-representations we look up the character values at 2B and 3B (see below for the first batch of those) and these together with the dimensions determine the dimension vector
For example take the 196883-dimensional irreducible. Its 2B-character is 275 and the 3B-character is 53. So we are looking for a dimension vector such that
Okay, so for each of the 194 irreducibles
and that the number of arrows from vertex
This data then determines completely the
But then, one doesn’t have to go for the full regular representation of the Monster. Any faithful permutation representation will do, so we might as well go for the one of minimal dimension.
That one is known to correspond to the largest maximal subgroup of the Monster which is known to be a two-fold extension
(in standard Atlas-ordering) and hence repeating the arguments above we get a quiver on just 9 vertices! The actual numbers of loops and arrows (I forgot to mention this, but the quivers obtained are actually symmetric) obtained were found after laborious computations mentioned in this post and the details I’ll make avalable here.
Anyone who can spot a relation between the numbers obtained and any other part of mathematics will obtain quantities of genuine (ie. non-Inbev) Belgian beer…
Leave a Comment