I’m trying to get into the latest Manin-Marcolli paper Quantum Statistical Mechanics of the Absolute Galois Group on how to create from Grothendieck’s dessins d’enfant a quantum system, generalising the Bost-Connes system to the non-Abelian part of the absolute Galois group
In doing so they want to extend the action of the multiplicative monoid
Here they use an idea, originally due to Jordan Ellenberg, worked out by Melanie Wood in her paper Belyi-extending maps and the Galois action on dessins d’enfants.

To grasp this, it’s best to remember what dessins have to do with Belyi maps, which are maps defined over
from a Riemann surface
Wood considers a very special subclass of these maps, which she calls Belyi-extender maps, of the form
defined over
The upshot being that post-compositions of Belyi’s with Belyi-extenders
The crucial Ellenberg-Wood idea is then to construct “new Galois invariants” of dessins by checking existing and easily computable Galois invariants on the dessins of the Belyi’s
For this we need to know how to draw the dessin of
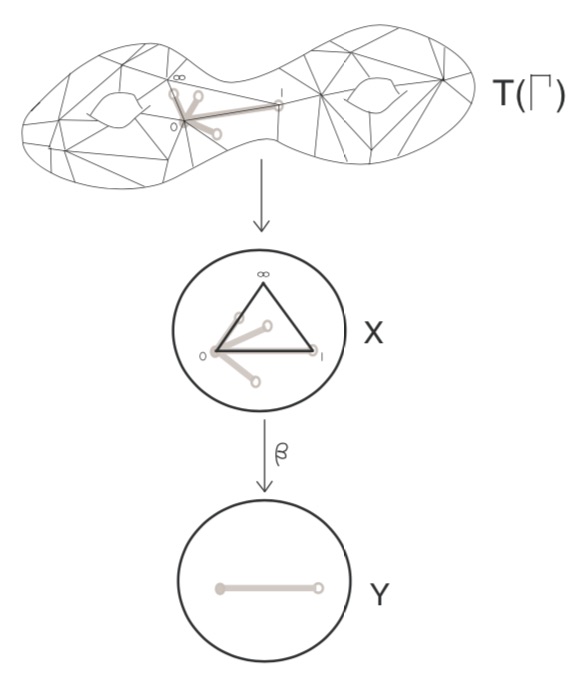
Here, the middle dessin is that of the Belyi-extender
One has to replace each of the black-white edges in the dessin of
Thus, a Belyi-expander
In her paper, Melanie Wood says she can separate dessins for which all known Galois invariants were the same, such as these two dessins,
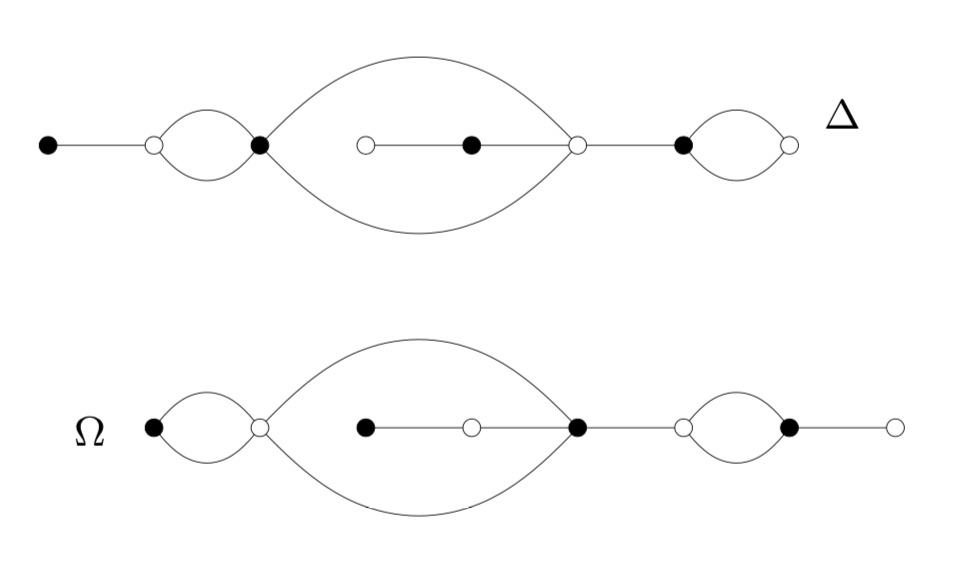
by inflating them with a suitable Belyi-extender and computing the monodromy group of the inflated dessin.
This monodromy group is the permutation group generated by two elements, the first one gives the permutation on the edges given by walking counter-clockwise around all black vertices, the second by walking around all white vertices.
For example, by labelling the edges of
Let’s inflate these dessins using the Belyi-extender
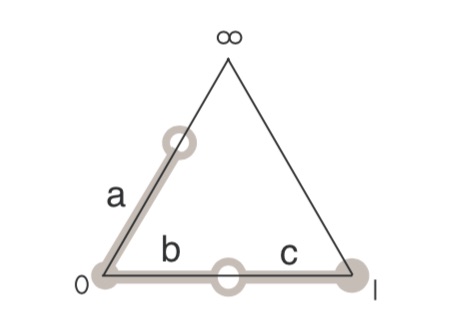
It took me a couple of attempts before I got the inflated dessins correct (as i knew from Wood that this simple extender would not separate the dessins). Inflated
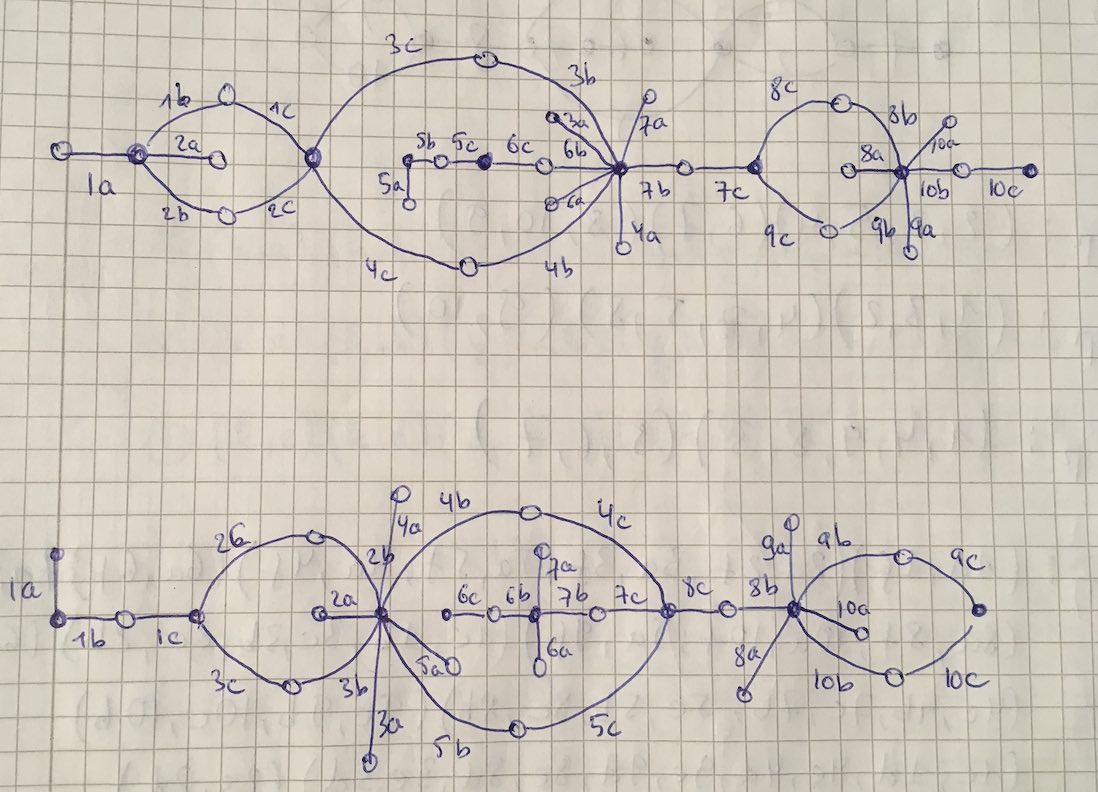
Both dessins give a monodromy group of order
Now we’re ready to do serious work.
Melanie Wood uses in her paper the extender
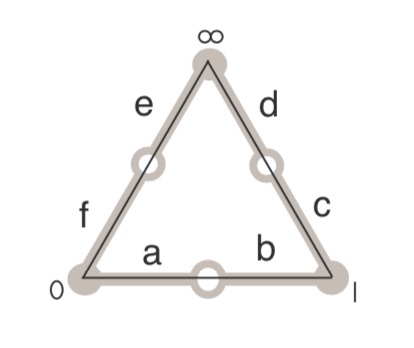
and says she can now separate the inflated dessins by the order of their monodromy groups. She gets for the inflated
It’s very easy to make mistakes in these computations, so probably I did something horribly wrong but I get for both
I’d be very happy when someone would be able to spot the error!