Here, MaMuTh stands for Mathematical Music Theory which analyses the pitch, timing, and structure of works of music.
The Elephant is the nickname for the ‘bible’ of topos theory, Sketches of an Elephant: A Topos Theory Compendium, a two (three?) volume book, written by Peter Johnstone.
How can we get as quickly as possible from the MaMuth to the Elephant, musical illiterates such as myself?
What Mamuth-ers call a pitch class (sounds that are a whole number of octaves apart), is for us a residue modulo
We’ll just denote them by numbers from
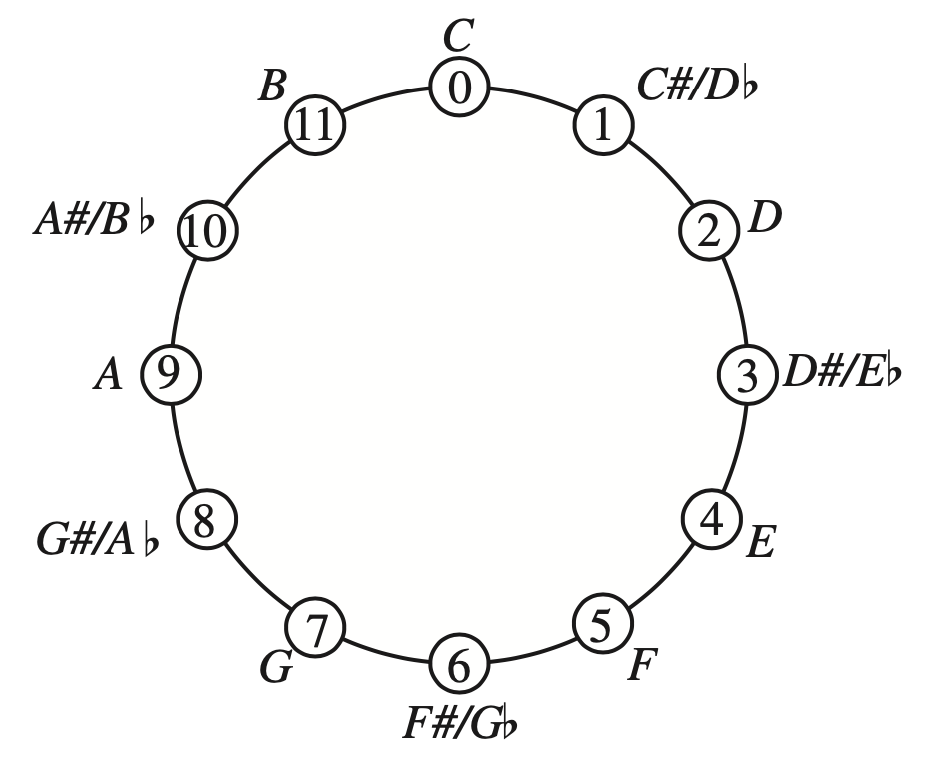
Our regular
and twelve reflexions, which they call involutions, given by
What for us is the dihedral group
Let’s move from individual notes (or pitch classes) to chords (or triads), that is, three notes played together.
Not all triples of notes sound nice when played together, that’s why the most commonly played chords are among the major and minor triads.
A major triad is an ordered triple of elements from
and a minor triad is an ordered triple of the form
where the first entry
For us, it is best to view a triad as an inscribed triangle in our regular
Starting from the root, and moving clockwise, we encounter in a major chord-triangle first the middle edge, then the small edge, and finally the large edge. For a minor chord-triangle, we have first the small edge, then the middle one, and finally the large edge.
On the left, two major triads, one with root
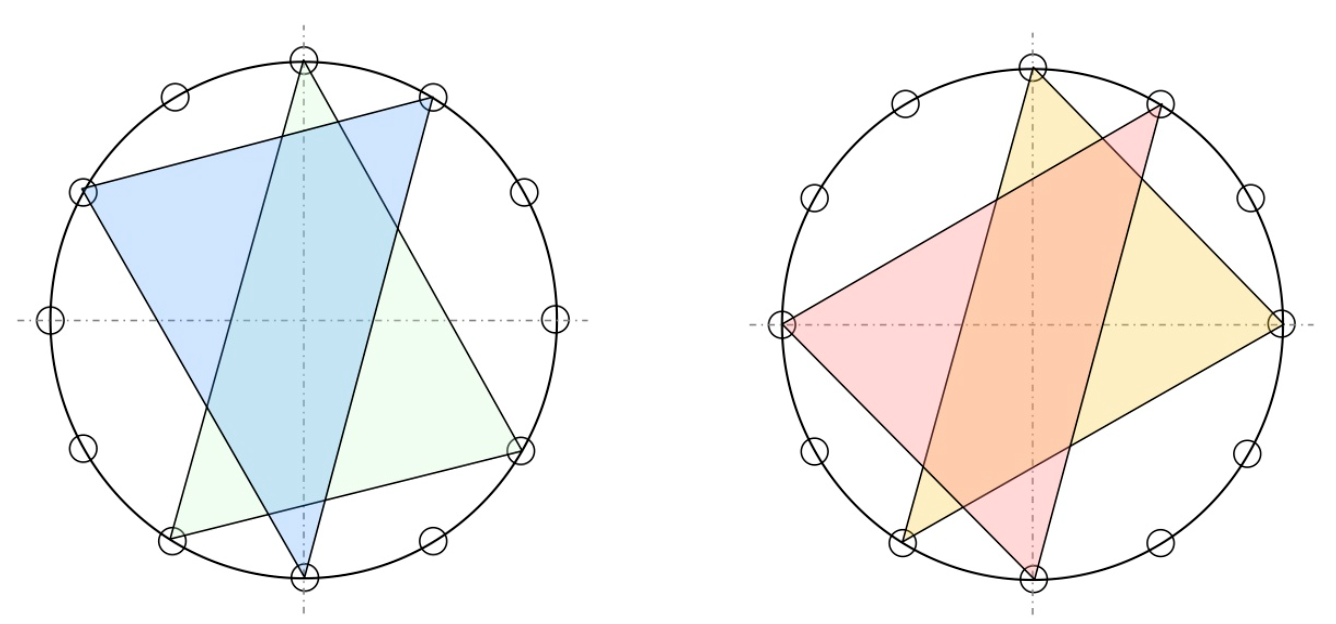
(Btw. if you are interested in the full musical story, I strongly recommend the alpof blog by Alexandre Popoff, from which the above picture is taken.)
Clearly, there are
From the shape of the triad-triangles it is also clear that rotations (transpositions) send major triads to major triads (and minors to minors), and that reflexions (involutions) interchange major with minor triads.
That is, the dihedral group
Can we hear the action of the very special group element
This action is not only the transposition by three full tones, but also a point-reflexion with respect to the center of the
In It’s
“The
Let’s move on in the direction of the Elephant.
We saw that the only affine map of the form
But, we can ask for the collection of all affine maps
A quick case-by-case analysis shows that there are just eight such maps: the identity and the constant maps
and the four maps
Compositions of such maps again preserve the set
gap> a:=Transformation([10,1,4,7,10,1,4,7,10,1,4,7]);;
gap> b:=Transformation([12,8,4,12,8,4,12,8,4,12,8,4]);;
gap> gens:=[a,b];;
gap> T:=Monoid(gens);
8
The monoid
The monoid
Actually, Noll considers just one such presheaf (and its sub-presheaves) namely
He is interested in the sheafifications of these presheaves with respect to Grothendieck topologies, so we have to describe those.
For any monoid category, the subobject classifier
Using the GAP sgpviz package we can draw its Cayley graph (red coloured vertices are idempotents in the monoid, the blue vertex is the identity map).
gap> DrawCayleyGraph(T);
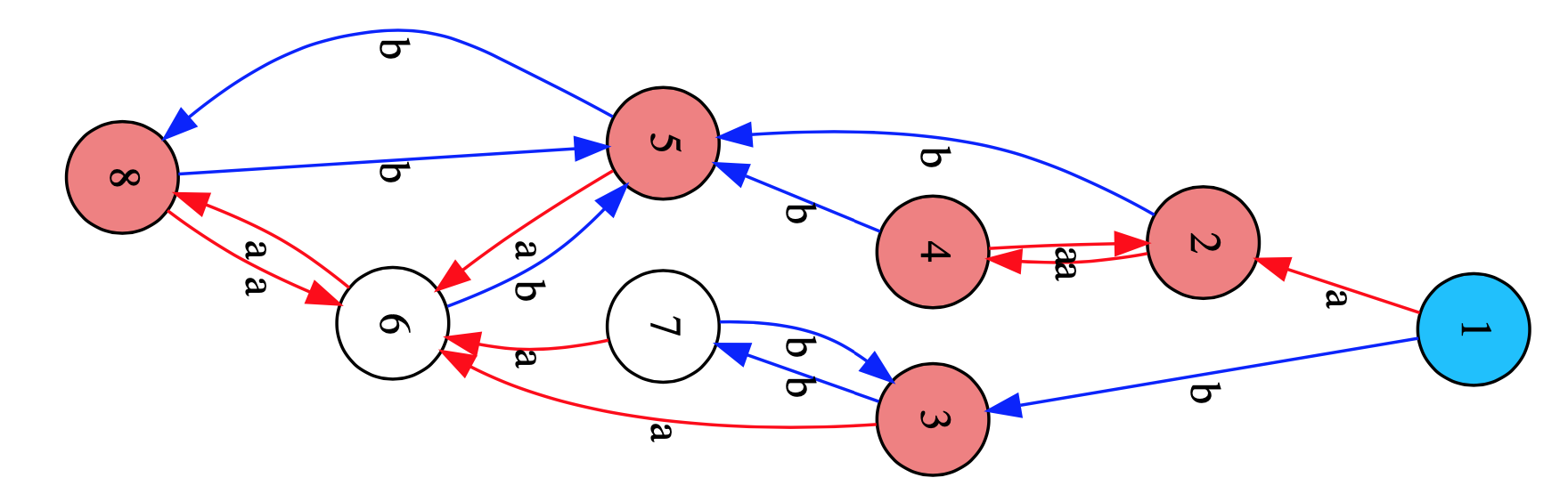
The elements of
This gives us that
As a subobject classifier
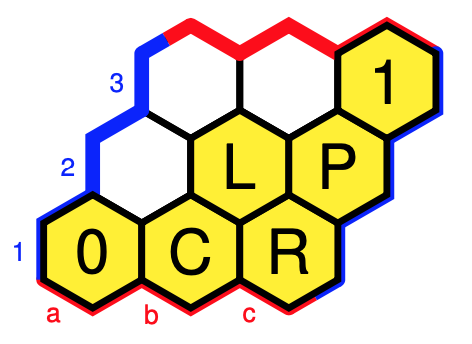
In this case, Grothendieck topologies coincide with Lawvere-Tierney topologies, which come from closure operators
- if
, then
Colouring all cells with the same
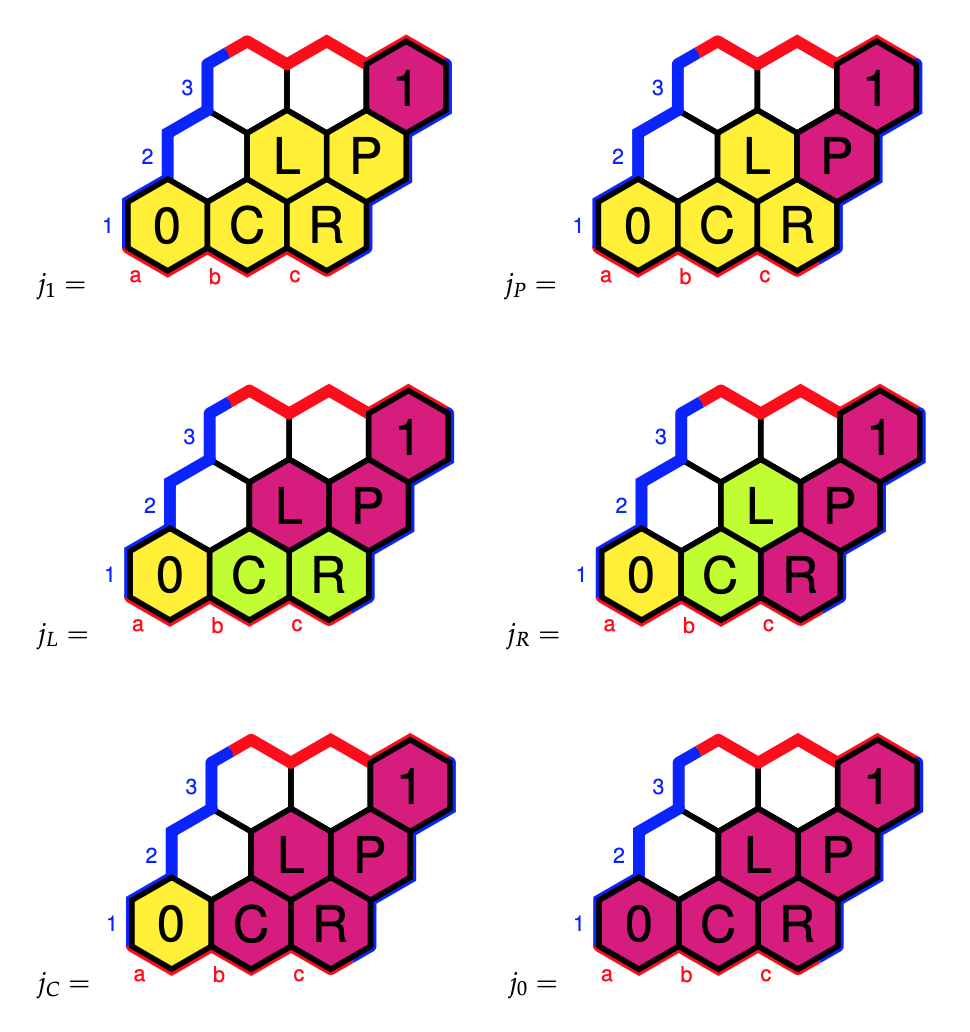
The triadic monoid
The point of the subobject classifier
What does the morphism
iff iff and are in iff but is not iff but is not iff neither nor is in
Remember that
a:=Transformation([10,1,4,7,10,1,4,7,10,1,4,7]);;
b:=Transformation([12,8,4,12,8,4,12,8,4,12,8,4]);;
so we see that
are mapped to is mapped to are mapped to are mapped to are mapped to
Finally, we can compute the sheafification of the sub-presheaf
The musically interesting Grothendieck topologies are
- For
we get the sheaf which Mamuth-ers call a Major-Minor Mixture as these are the notes of both the major and minor -triads - For
we get which is an example of an Hexatonic scale (six notes), here they are the notes of the major and minor and -triads - For
we get which is an example of an Octatonic scale (eight notes), here they are the notes of the major and minor and -triads
We could have played the same game starting with the three notes of any other major triad.
Those in the know will have noticed that so far I’ve avoided another incarnation of the dihedral
(to be continued…)
Comments closed